Four main causal pathway groups have been defined in the Assessment for grouping the hazards from coal resource development. For changes to surface water the causal pathway groups are:
- ‘Surface water drainage’
- ‘Operational water management’.
For changes to groundwater flows the causal pathway groups are:
- ‘Subsurface depressurisation and dewatering’
- ‘Subsurface physical flow paths’.
Table 14 provides a summary for each causal pathway.
Table 14 Causal pathway groups and the associated hazards for the Hunter subregion
Data: Bioregional Assessment Programme (Dataset 1)
Water near, and at, the land surface most often occurs in very thin flows. The direction and velocity of surface flows is controlled primarily by local land gradient, and for river flows a combination of the water surface gradient and flow depth. Surface roughness also affects flow velocity (e.g. vegetation cover density and size, material at the surface, presence of other debris). Any natural or anthropogenic activity that changes the roughness, local slope, local flow direction, vegetation or surface material can affect surface flow direction and velocity. Groundwater flows are primarily controlled by differences in local groundwater elevation, as the saturated thickness becomes large. The flow velocity and direction is locally controlled by hydraulic conductivity of rocks and strata, and its anisotropic properties, and on a larger scale by heads and flows at boundaries (e.g. rivers and surface water bodies, oceans, geological barriers and facies changes, diffuse rainfall recharge and evaporation). Anthropogenic activities, such as point recharge from irrigation or point extraction by pumping, change gradients locally and affect the direction and velocity of flows within a groundwater system.
Most water fluxes occur at the surface and in near-surface rock layers. In general, the groundwater flow paths follow the subregion’s topographic directions from the uplands toward the central valley and then to the ocean, with the Goulburn and Hunter river valleys being regional flow discharge zones. Surface porous and fractured rock layers contain the local groundwater flow paths, and many provide baseflow to streams, rivers and springs (McVicar et al., 2015). Inflow from saline streams and groundwater discharge strongly influence the quality of the Hunter River in medium-to-low flows (Kellett et al., 1989). Under high-flow conditions industrial discharge from coal mining and power generation activities, as controlled by the Hunter River Salinity Trading Scheme (HRSTS), may increase river salinity within legislated bounds (McVicar et al., 2015, p. 103).
Open-cut mines operate extensively at the land surface, but may extract coal from depths of tens or hundreds of metres, and may require digging through multiple geological layers, some of which may be part of an aquifer or aquitard. From the surface water perspective, rain that falls within the working area is considered produced water, and must therefore be retained on site. Water that flows through the working area must be redirected, or retained on site as produced water. These surface water causal pathways result in hazards that disrupt natural drainage, which may lead to reductions in surface water flows feeding streams or water-dependent ecosystems, or increase erosion of surface soils.
Associated with open-cut pits is the requirement for dewatering to allow removal of overburden and coal. The amount of water requiring removal will vary by the size of the mine pit, the specific aquifer layers that have been cut through, and the hydraulic properties of the material in those layers and in the base of the pit. Removing water from these layers results in a reduction of head in the layer, which may change groundwater gradients and induce flow away from rivers and streams, and other surface water features.
Mine operations may require the removal of water from surface streams or bodies for processing or general mine-site operations. To compensate for this, mines are required to obtain equivalent credits from water licences. A hazard will arise if there is sufficient gradient induced that water in the stream is drawn towards a mine operation, and it affects the flow duration in the watercourse. A hazard is also generated with mine-water disposal to streams that may contain a high level of salt or other chemicals. From the view of river salinity, such discharges are controlled by the HRSTS and not in the scope of the hydrological modelling for this BA, but will be part of the narrative.
Underground mines have the same causal pathway groups as open-cut mines (i.e. ‘Subsurface depressurisation and dewatering’ and ‘Subsurface physical flow paths’), although they may differ in their severity. In addition, underground mines may cause subsidence, with changes to the physical structure and properties of rocks and sediments between the mining plane and some distance up to, and including, the land surface. Where land subsidence coincides with a natural stream or river, water may be lost from the stream temporarily or permanently.
2.3.5.3.1 Open-cut and underground coal mines
Figure 23 illustrates some of the mine activities for the causal pathway groups associated with open-cut and underground coal mines, which are discussed in the following sub-sections.
Figure 23 Causal pathways associated with open-cut and underground coal mining
ROM = run of mine
‘Surface water drainage’ causal pathway group
The ‘Surface water drainage’ causal pathway group pertains to mine hazards that physically disrupt the surface topography and thereby alter the flow of surface water within and from the mine site. Figure 23 illustrates some of the mining activities that can affect surface water drainage, including excavation of pits; diversion of water around mine-site operation areas with drains or walls (C in Figure 23); and construction of dams to store tailings, mine water and catchment runoff as part of ‘Operational water management’ causal pathway group (D in Figure 23). Figure 24 summarises the key hydrological changes. Changes to surface water drainage can occur not just from altering the surface topography but also through changing the infiltration properties at the land surface, causing changes in diffuse recharge and potentially changes in streamflow elsewhere in the catchment. Disruptions can cause changes to the quality and total amount of water flowing over the land surface.
Figure 24 'Surface water drainage' causal pathway group
The Δ symbol indicates a change in the quantity, but not a direction of change.
WQ = water quality
Direct rainfall and runoff generated on a mine site are retained on site. This water that would naturally contribute to surface water drainage networks represents a loss to the river system. While the volume of water may be relatively small if it occurs adjacent to a major stream or river, on smaller upland creeks the volumes may become significant. Any secondary value this runoff may provide, such as infiltration further downslope, will be lost.
Intercepting runoff or streamflow into a mine area will remove a volume of water from the larger surface water drainage system. Both the quantity and quality of water downstream may be affected; quality may improve if the runoff or flow was naturally saline or highly turbid, or decrease if the inflow was fresher than the main stream. Surface water that is diverted around a mining operation may lead to concentration of surface flows that may negatively affect water quality due to increased erosion and turbidity.
The subsidence that results from underground mining can affect both surface drainage and infiltration properties. The process of subsidence is a lowering of the land surface due to collapse of the regolith above an underground mine (Holla and Barclay, 2000). It occurs in two phases: active and residual. The active phase comprises about 90% of total subsidence and follows the advance of the mining front, occurring almost immediately with roof collapse. The residual phase takes more time, and occurs as the rocks compress and adjust in the collapsed zone above the mining void.
The collapsed zone (B in Figure 23) is highly fractured and often has enhanced hydraulic conductivity and storage to a thickness of about five times the thickness of the extracted seam, although cracking and some increased hydraulic conductivity can occur over a thickness of 20 to 30 times the extracted seam height depending on the strength of overlying strata (ACARP, 2002). At the surface, longwall mining creates long closed rectangular basins that extend beyond the physical boundaries of the mined area. Depending on depression depth, orientation, gradient of depression and connectivity to drainage lines, these depressions will retain more runoff than pre-disturbance and can be associated with local waterlogging. Under irrigated cropping lands, these changes have been identified as potentially impacting crop yields and disrupting water supply channels (Cotton Australia, 2013). In the Hunter subregion, potential loss of streamflow from fracturing of stream beds appears to be a bigger concern. Shallow longwall mining (e.g. less than 100 m below surface) can result in total loss of flow in smaller streams, while a deeper mine may cause only limited or temporary losses (OEH, 2013). In the Hunter Valley, Eui Creek, Wambo Creek, Bowmans Creek, Fishery Creek and Black Creek have all been affected by subsidence due to underground coal mining. Damage has occurred in the form of increased streambed erosion leading to degraded water quality, loss of streamflow and death of riparian vegetation. Extraction plans, prepared as part of the development consent process for longwall mines in NSW, must identify water resources at risk from the proposed mining and detail how mining will be undertaken to minimise impacts.
Longwall mining occurs adjacent to and under Lake Macquarie and has contributed to localised lowering of the lake bed and foreshore. Mining operations in the vicinity of the lake are controlled by NSW Department of Industry Resources and Energy to limit the maximum vertical subsidence within a high water mark subsidence barrier to 20 mm, which is the effective limit of measureable subsidence. This limit has been imposed to protect the shores of Lake Macquarie from inundation in response to past mining induced damage to the shoreline and adjacent waterfront homes, such as occurred at Chain Valley Bay in 1986. In addition, a habitat protection plan has been developed under the NSW Fisheries Management Act 1994 to protect seagrasses in NSW. Seagrass beds occur in shallow subtidal waters of Lake Macquarie and are sensitive to base-level changes from mine subsidence. Their density and health are tied to light penetration, which decreases exponentially with increasing water depth (Baird et al., 2003). Extraction plans for mines going under the lake are required to limit the impact of subsidence within the Lake Macquarie seagrass protection barrier.
‘Operational water management’ causal pathway group
This causal pathway group relates to the creation or modification of water management systems that facilitate sourcing, storing, reuse and release or disposal of water within the mine site (D in Figure 23). This water may be derived from rainfall, runoff, streamflow, pit dewatering or any other operation or process within the mine site. Figure 25 summarises the pathways in the ‘Operational water management’ causal pathway group that have off-site effects, but do not include intra-site water flows that might be a part of site water management (e.g. mine water make held in dams on site).
Figure 25 'Operational water management' causal pathway group
The Δ symbol indicates a change in the quantity, but not a direction of change.
WQ = water quality
Clearly any release of water to the surface water drainage network, or a surface water feature, will increase the total amount of water and may affect the water quality (see Figure 25). For salt concentrations related to mine water releases in the regulated Hunter River alluvium, the process is covered by the HRSTS and is not in the scope of this BA. Monitoring the water quality for other contaminants, such as heavy metals or BTEX (benzene, toluene, ethylbenzene and xylene), and their removal or disposal is the responsibility of individual mining companies under their mine water management agreements. Any release of contaminated water to the surface water system, for example by leakage or overflow from a storage dam, may negatively affect downstream water quality.
‘Subsurface depressurisation and dewatering’ causal pathway group
Mine dewatering is an operation that lowers groundwater levels so that extractive mining can proceed (A in Figure 23). This can change groundwater pressure gradients due to head changes, or other gradients such as temperature, density and chemical composition. The largest pressure gradient changes will occur in the coal seams that are targeted for mining, either near the surface or in longwall panels.
This causal pathway group leads to a hazard that is a degradation of the water resource in terms of availability or quality in the surface water system, and conjunctively in the river/alluvial aquifer. In the case of direct extraction from streams it is necessary for mine operations to obtain credits to extract this water, and conditions may be placed on such extraction at times of low flow, for example. Where water can be extracted from an alluvial aquifer and the stream is well connected with local groundwater, then prolonged extraction from the aquifer may result in enhanced leakage from the stream, and therefore affect total streamflow (see Figure 26a). Under the Hunter Water Sharing Plan for the Hunter Unregulated and Alluvial Water Sources (DWE, 2009) there are specific rules under low- and no-flow conditions to prevent pumping that may stress the alluvial aquifer under drying conditions.
Similarly, lowering the groundwater level in surface layers that are being dewatered or mined, can induce a gradient away from a connected alluvial aquifer. If water levels are maintained in the alluvial aquifer then water must be sourced from the river (see Figure 26b). If that source is unable to maintain the required flux, then water levels in the alluvial aquifer will ultimately be reduced. The water quality and groundwater levels in the alluvial aquifer will be resolved by the complexities of the pathways and interactions between the potential source (e.g. the river), and the sink (e.g. the depressurised local rock layer). AGEC (2013) provides an example at Mount Arthur Coal Mine of changing the direction of the groundwater gradient toward Permian rocks and away from the Hunter River alluvial aquifer through depressurisation, but without a change to water levels in the alluvial aquifer.
The Δ symbol indicates a change in the quantity, but not a direction of change.
WQ = water quality
‘Subsurface physical flow paths’ causal pathway group
This causal pathway group shares many similarities with the ‘Subsurface depressurisation and dewatering’ causal pathway group, as can be seen by comparing Figure 26b and Figure 27. While the former applies to water losses or gains within the stream plus alluvial aquifer system, this causal pathway group relates to water transfer between any layers. This causal pathway group involves physical modification of the structure of the rock strata that leads to new, or changes to existing, pathways that water can potentially move through (such as at B in Figure 23). Note that the creation of a pathway does not necessarily mean water will flow through it; fluxes are governed by the pressure gradients. Off-site effects can result due to changes in baseflow to nearby streams or changes in discharge to the land surface, such as via springs or wetlands (Figure 27).
Where aquifer layers, or indeed the land surface, are separated by aquitards or other low-conductivity barriers, the water within each aquifer may have different chemical properties and constituents. Mixing of water between layers may be undesirable due to contamination of a resource by lower quality water, or by drainage to another layer leading to reduced availability, or loss, of a resource. Reduced resource availability due to water removed by a newly connected aquifer or pathway is analogous to the previously described ‘Subsurface depressurisation and dewatering’ causal pathway group.
Hazards in this causal pathway group can be generated by drilled holes for exploration, monitoring or dewatering (A in Figure 23) and by excavation of open-cut and underground mines. This hazard may be generated by wells due to inadequate design or completion of a hole, mechanical failure of the well casing or sealants, or by failure induced by movement of the layers the well is drilled through. Department of the Environment (2014) provides a thorough review of the leakage issues and best practices associated with bore construction in Australia. Physical flow paths created or enhanced through well construction are not represented in the BA numerical modelling: the connections are point scale, and are unlikely to result in significant changes in groundwater flows, and therefore will not affect regional watertables.
Figure 27 'Subsurface physical flow paths' causal pathway group
The Δ symbol indicates a change in the quantity, but not a direction of change.
WQ = water quality
Through the excavation of open-cut and underground mines, physical pathways can be created between aquifers that were previously separated. In longwall mining, the inevitable collapse of overburden layers into the mine void following coal extraction leads to subsidence. The collapsed zone is characterised by fracturing and hydraulic enhancement from changes in horizontal and vertical compression and tension. The hydraulic enhancement varies with depth, with large increases in permeability associated with the caved and fractured zones immediately above the worked area, diminishing through the constrained and surface zones. It also varies between the goaf area (above the worked area) and the rib area, which extends out from the worked area. As already discussed, where fracturing coincides with drainage lines at the surface, streamflow losses can occur. Thus longwall mining leads to creation and enhancement of subsurface physical pathways, aquifer depressurisation and modifications to surface water drainage.
Furthermore, enhancing connectivity means greater potential for water-borne pollutants to move through and between aquifers and can also mean enhanced weathering. Sulfide-bearing minerals that are exposed to both oxygen and water can create sulfuric acid, and the resulting drainage water is termed ‘acid rock drainage’ (Blodau, 2006). This water may contaminate surface water bodies by escaping storage dams overland or via newly connected underground pathways, or form on the face of mine walls and acidify water in mining voids. There are several inactive mine sites in the lower Hunter that are leaking, or have leaked, acid rock drainage to surface watercourses at Aberdare East, Testers Hollow, Neath, Dagworth, Greta and Rothbury (SMH, 2013).
The spatial and temporal extents of hydrological changes from open-cut and underground mining are summarised by causal pathway group in Table 15. The temporal extent of, for example, discharge to streams and extraction from streams as part of the ‘Operational Water Management’ causal pathway group is measured in years, but likely only over the life of the mine, as these activities are most closely associated with a producing mine. Life of mine and post-mining hydrological effects of land modification due to hazards in the ‘Surface water drainage’ causal pathway group will depend on land management and mine rehabilitation. The surface water characteristics post-rehabilitation may be altered with respect to infiltration, runoff production and vegetation health. The effects of subsidence on surface water drainage can be permanent. Groundwater modelling has indicated that filled-in mining voids and lake pits can be a groundwater sink for decades or centuries (Vandenburg, 2011; McCullough et al., 2012), and that mine closure is a whole-of-landscape development.
‘Vertical’ is included in the ‘Spatial extent’ column for some causal pathway groups in Table 15. It indicates that effects propagate both laterally, as aquifer properties and connectivity allow, and vertically, potentially through several different strata and potentially to the land surface for underground mining.
Table 15 Spatial and temporal extent of open-cut and underground mining by causal pathway groups
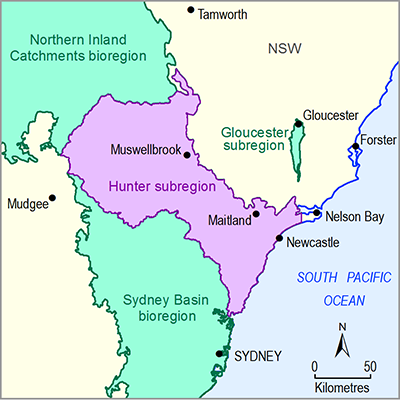
Product Finalisation date
- 2.3.1 Methods
- 2.3.2 Summary of key system components, processes and interactions
- 2.3.3 Ecosystems
- 2.3.4 Baseline and coal resource development pathway
- 2.3.5 Conceptual modelling of causal pathways
- Citation
- Acknowledgements
- Currency of scientific results
- Contributors to the Technical Programme
- About this technical product